PROTEIN
Protein is a vital nutrient in nutrition and health, and its significance cannot be overstated. The Greek word used to describe this nutrient, “proteios,” means “primary,” “in the lead,” or “standing in front,” highlighting its importance. Proteins are present throughout the body, with the majority located in skeletal muscle (over 40%), body organs (over 25%), and the remainder mainly in the skin and blood.
Proteins are essential for good nutrition due to their constituent amino acids. The body requires these amino acids to create various proteins and nitrogen-containing molecules necessary for life. Each protein found in the body is distinct in the sequence pattern and characteristics of its constituent amino acids. Amino acids can be classified based on their structure, net charge, polarity, and essentiality, among other factors.
Table of Contents
- Amino Acid Classification
- Amino Acid Biochemistry
- Essential & Non-Essential Amino Acids
- Protein Biochemistry
- Sources Of Amino Acids
- Protein Digestion
- Protein Absorption
- Amino Acid Metabolism
- Energy Production
- Ketone Body Production
- Other Uses Of Amino Acids
- Protein Synthesis
- Functional Roles Of Protein
- Nitrogen-Containing Non-Protein Compounds
- Glutamine Metabolism
- The Alanine-Glucose Cycle
- Skeletal Muscle
- Protein Myths
- Meal Frequency
- Summary
Amino Acid Classification
Structurally, amino acids have a central carbon (C), at least one amino group (—NH2), at least one carboxy (acid) group (—COOH), and a side chain (R group) that makes each amino acid unique.
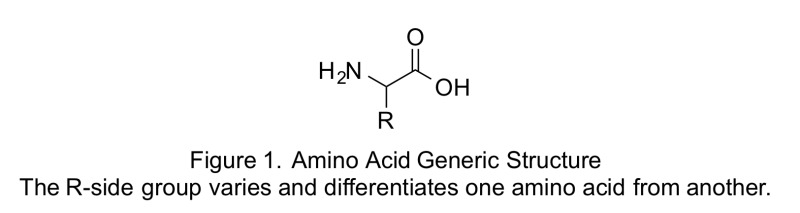
Depending on the pH of the environment, the amino and carboxy groups can accept or donate H1, and thus the amino group may be represented as 1NH3 and the carboxy group as COO2. The distinctive characteristics of the side chains of the amino acids that make up a polypeptide bestow on a protein its structure and influence its functional role in the body. Amino acids can differ in structure in environments with different acidity levels.
These same distinctive characteristics determine whether certain amino acids can be synthesized in the body or must be ingested, as well as which metabolic pathways of the body the amino acids can enter.
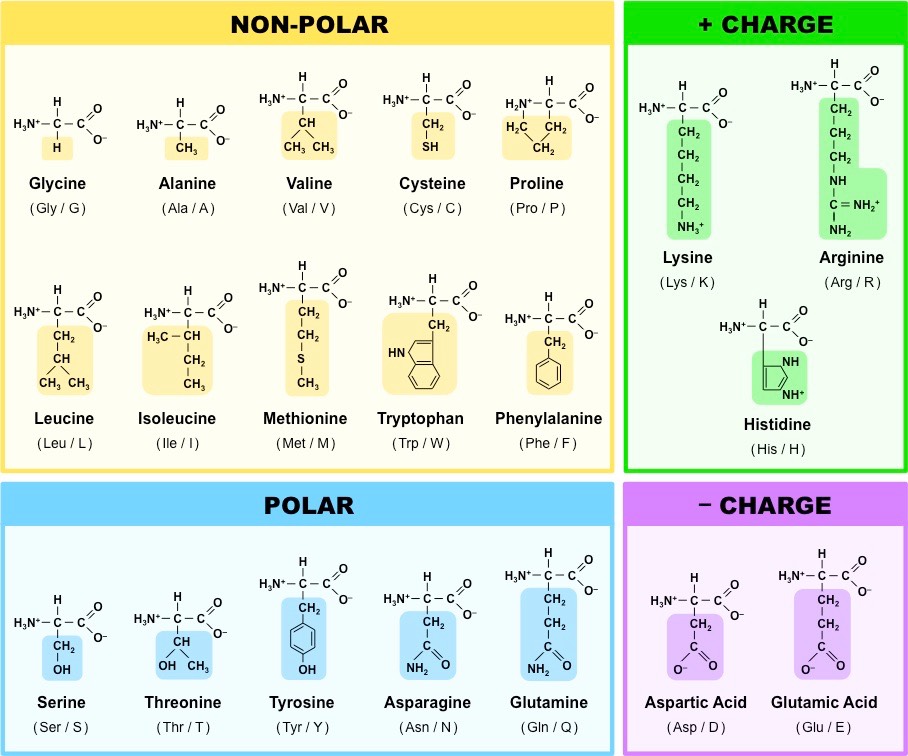
Amino Acid Biochemistry
Amino acids can be classified according to their polarity, which refers to their tendency to interact with water at physiological pH. The polarity of an amino acid is determined by its R group or side chain. While some amino acids are either polar or nonpolar, others have varying degrees of polarity.
The polar-charged amino acids include the dicarboxylic amino acids (aspartic acid and glutamic acid) and basic amino acids (lysine, arginine, histidine). These amino acids interact with water, form salt bridges, and interact with electrolytes and minerals like potassium, chloride, and phosphate.
Neutral amino acids can be polar, nonpolar, or relatively nonpolar. The side chains of polar neutral amino acids contain functional groups that can form hydrogen bonds with water. These amino acids are often found on the surfaces of proteins or oriented inward to function at a protein’s binding site.
In contrast, nonpolar or hydrophobic amino acids do not interact with water. The aromatic amino acids are relatively nonpolar, and while they can form limited hydrogen bonds with water, they do not interact with water to the same extent as polar amino acids. Nonpolar amino acids are typically found tightly coiled or compacted and oriented toward or within the central region or core of proteins.
Essential & Non-Essential Amino Acids
While chemists classify amino acids based on their structure and properties, nutritionists focus on their essentiality in the body. Essential amino acids cannot be synthesized by the body and must be obtained from the diet. The 9 essential amino acids are:
- leucine,
- isoleucine,
- valine,
- lysine,
- tryptophan,
- threonine,
- methionine,
- phenylalanine and
- histidine.
However, this classification as either essential or nonessential is not always flexible enough to account for changing physiological circumstances. A third category, called conditionally or acquired indispensable amino acids, exists to address this issue. Under certain conditions, dispensable amino acids may become indispensable. For example, if precursor availability is limited or if an organ is not functioning properly, amino acid metabolism may not proceed normally, causing a dispensable amino acid to become essential.
Protein Biochemistry
The 20 natural amino acids are proteinogenic, protein building. Amino acids strung together form peptidide chains that are in turn incorporated into proteins.
- Peptide is a short amino acid chain.
- Dipeptide: a peptide consisting of 2 amino acids.
- Tripeptide: a peptide consisting of 3 amino acids.
- Peptides with 2-20 amino acids are called oligopeptides.
- Peptides with more than 20 amino acids are called polypeptides.
These amino acids are folded into shape by the ribosomes to create complex structures.
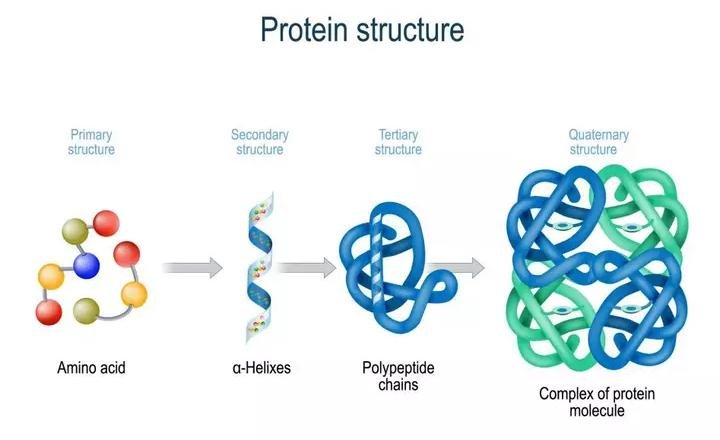
Proteins have 4 levels of structure:
- The primary structure of a protein represents the amino acid sequence of the protein.
- The secondary structure is the coiling, folding and bending of the protein. This shape is created by i.a. hydrogen bonds, which are electrical attractions that keep atoms together and electrostatic/ionic attractions between amino acids (akin to magnets).
- The tertiary structure of protein is the overall or total three-dimensional configuration of the protein. This is basically the resulting total shape of the combined secondary structure shapes attached together.
- Some proteins also have a quatarnary structure as a result of interacting polypeptide chains that form a so called oligomer.
Sources of Amino Acids
Amino acids are derived from protein. Both dietary (exogenous) and endogenous proteins provide the body with amino acids. Dietary sources of protein include:
- Animal products such as meat, poultry, fish, eggs, and dairy (with the exception of butter, sour cream, and cream cheese)
- Plant products such as grains, grain products, legumes (including lentils, beans, and peas), nuts, seeds, and vegetables.
Following ingestion, exogenous proteins serve as sources of the essential amino acids, nonessential amino acids, and additional nitrogen needed to synthesize more nonessential amino acids, nitrogen-containing compounds, and protein in the body.
Endogenous proteins presented to the digestive tract represent another source of amino acids and nitrogen. Endogenous proteins include:
- Desquamated mucosal cells
- Digestive enzymes and glycoproteins
The digestive enzymes and glycoproteins are derived from secretions of the salivary glands, stomach, intestine, liver, and pancreas. The digestive tract’s mucosal cells contain a variety of proteins (such as apoproteins, structural proteins, and cytosolic enzymes) that when sloughed into the gastrointestinal (GI) tract are degraded. Most of these endogenous proteins, which typically total 70 g or more per day, are digested and provide amino acids that are available for absorption. Digestion of these proteins and the absorption of subsequently generated amino acids are crucial for protein nutriture.
That this is not “free protein” that you need to substract from your total protein need, as ultimately the building blocks of these proteins still have to be consumed in some form. Ultimately, your body derives all of the building blocks it needs to create proteins from exogenous sources, notably the consumption of food.
Protein Digestion
Protein digestion commences in the stomach, where the gastric juice, containing hydrochloric acid (HCl), is responsible for denaturation of the quaternary, tertiary, and secondary protein structures. The acidic environment, with a gastric pH less than 3, can break apart electrostatic and hydrogen bonds to unfold the protein. However, the peptide bonds remain unaffected by hydrochloric acid.
Pepsinogen, secreted by the gastric chief cells as an inactive enzyme or zymogen, is activated by hydrochloric acid to pepsin. Pepsin can then function as an endopeptidase, meaning it hydrolyzes interior peptide bonds within proteins or polypeptides, at a pH of approximately 3.5. Pepsin exhibits low specificity and can attack peptide bonds adjacent to the carboxy end of a wide variety of amino acids, including pepsinogen and other proteins.
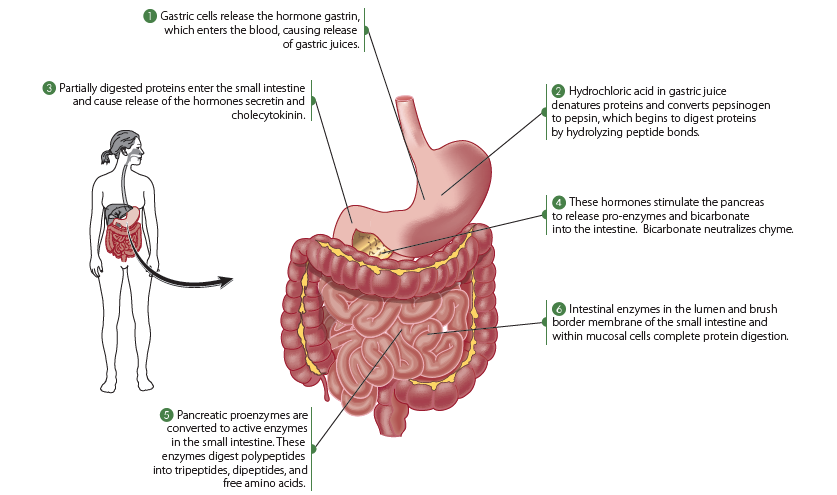
The end products of gastric protein digestion include primarily large polypeptides, along with some oligopeptides (short chains of amino acid peptide bonded to each other) and free amino acids. These end products subsequently enter the small intestine for further digestion.
Protein digestion yields two main end products: peptides (principally dipeptides and tripeptides) and free amino acids. To be used by the body, these end products must next be absorbed.
Protein Absorption
Absorption is the process that transports the end products of digestion from the lumen of the gastrointestinal (GI) tract, usually the small intestine, into the body. Amino acids are absorbed along the entire small intestine, but most are absorbed in the duodenum and proximal jejunum. The process of amino acid absorption into enterocytes requires carriers, which are integral membrane proteins. However, in rare situations in which large volumes of hypotonic fluids containing some amino acids have been ingested, absorption via passage through the tight junctions of enterocytes or transcellular endocytosis can occur.
The transport systems responsible for carrying amino acids across the brush border membrane into the intestinal cell are mainly sodium-dependent transporters. The affinity of a carrier for an amino acid depends on the hydrocarbon mass of the amino acid’s side chain and the net electrical charge of the amino acid. As the hydrocarbon mass of the side chain increases, affinity also increases. Neutral amino acids are absorbed at higher rates than basic or acidic amino acids. Essential amino acids are absorbed faster than nonessential amino acids, with methionine, leucine, isoleucine, and valine being the most rapidly absorbed.
Ingesting large quantities of one amino acid or a particular group of amino acids that use the same carrier system may create competition among the amino acids for absorption, impairing the absorption of the other, less concentrated amino acids carried by that same system. Therefore, amino acid supplements may result in impaired or imbalanced amino acid absorption.
The absorption of peptides is generally more rapid than that of an equivalent mixture of free amino acids. Although peptides compete with one another for transporters, peptide transport appears to occur more rapidly than amino acid transport and is thought to be the primary means by which most amino acids enter the intestinal cell.
Peptides, once within the enterocytes, are generally hydrolyzed by cytosolic peptidases to generate free intracellular amino acids. Intact peptides can be found occasionally in circulation, and this is thought to result from entry of peptides into the body via paracellular routes, which is more likely to occur in people with gastrointestinal tract disorders that affect intestinal permeability.
For amino acids to enter the blood and be used by other body tissues, they must be transported across the basolateral (serosal) membrane of the enterocyte and into interstitial fluid, where they enter the blood through capillaries of the villi for transport into the portal vein leading to the liver. The carriers found in the enterocyte’s basolateral membrane are generally sodium independent and similar to those found in other cell membranes of the body. Once absorbed into the bloodstream, amino acids are taken up by various organs, including the liver and kidneys, through carrier systems similar to those found in the intestinal cell membranes.
Amino Acid Metabolism
The liver is the primary site for the uptake of most amino acids following ingestion of a protein-containing meal. The liver is thought to monitor the absorbed amino acids and to adjust the rate of their metabolism (including catabolism or breakdown of amino acids and anabolism or use of amino acids for synthesis) according to the needs of the body.
Amino Acid Catabolism
Amino acid catabolism varies in different tissues during both fasting and the postprandial period. The liver has a high capacity for amino acid uptake and catabolism, taking up around 50-65% of amino acids from portal blood after a meal. The liver primarily catabolizes indispensable amino acids, except for branched-chain amino acids, which are mainly utilized by muscle and other organs like the heart.
Periportal hepatocytes in the liver catabolize most amino acids except for glutamate and aspartate, which are metabolized to a greater extent by perivenous hepatocytes. A significant portion of the liver’s energy (up to 50% of ATP) comes from amino acid oxidation. The energy produced is utilized for various purposes such as gluconeogenesis or urea synthesis, depending on the body’s nutritional state. The catabolism of amino acids involves transamination and/or deamination of amino acids, followed by the disposal of ammonia.
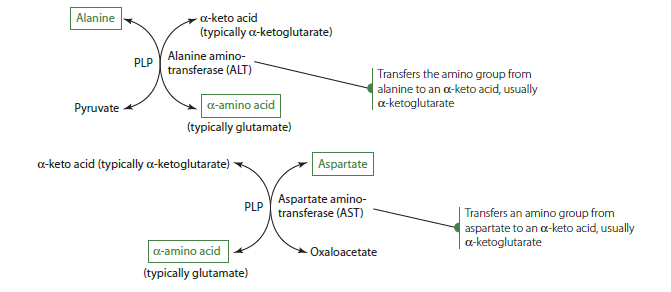
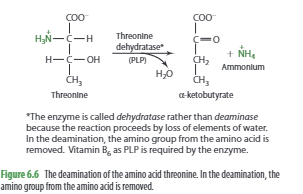
Amino acid catabolism typically begins with the removal or transfer of an amino group, although this is not always the case. The initial step usually involves either transamination or deamination. In transamination, the amino group from one amino acid is transferred to an alpha-keto acid, which becomes an amino acid, while the original amino acid is converted into an alpha-keto acid. This process is crucial for the synthesis of dispensable amino acids in the body.
The urea cycle is a vital process that occurs in the liver and is responsible for eliminating ammonia and ammonium ions from the body. Urea is produced by combining one nitrogen from ammonia, which is generated from the deamination reaction, and another nitrogen from aspartate, which comes from transamination. The carbon component of urea is derived from carbon dioxide or bicarbonate ions.
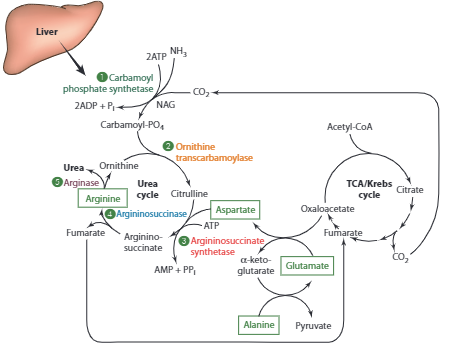
After urea is formed, it is usually transported in the bloodstream to the kidneys for excretion in urine, although up to 25% may be secreted into the intestine where it may be converted into ammonia by gut bacteria. The activity of urea cycle enzymes is influenced by diet and hormones, with low-protein diets or acidosis leading to reduced urea synthesis and urinary excretion of urea nitrogen. In healthy individuals with normal protein intake, blood urea nitrogen levels typically range from 8-20 mg/dL, with urinary urea nitrogen representing around 80% of total urinary nitrogen.
Defects in genes coding for urea cycle enzymes can result in hyperammonemia, with high levels of ammonia in the blood, and require a protein-restricted diet. However, the carbon skeletons of amino acids are not just waste products but have multiple potential uses within the cell. Depending on the specific amino acid and the body’s physiological and nutritional state, amino acid carbon skeletons can be used to generate energy, glucose, ketone bodies, cholesterol, and fatty acids.
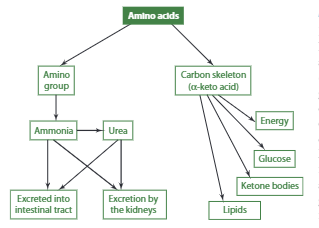
Energy Production
When the body doesn’t receive enough energy from the diet, it can use amino acids to produce energy. This process is called gluconeogenesis, and it involves using the carbon skeletons of certain amino acids to create glucose. Some examples include aspartate’s carbon skeleton (oxaloacetate) and alanine’s carbon skeleton (pyruvate) to produce glucose in cells. Other amino acids like glycine, serine, cysteine, tryptophan, and threonine can also be converted to pyruvate, while valine and methionine can yield succinyl-CoA.
Essentially, all natural amino acids except for lysine and leucine can be used to synthesize glucose. It’s important to note that for an amino acid to be considered glucogenic, its breakdown must yield pyruvate or TCA cycle intermediates.
Ketone Body Production
For an amino acid to be considered ketogenic, the catabolism of the amino acid must generate acetyl-CoA or acetoacetate, which are used for the formation of ketone bodies (also referred to as ketones). Some amino acids are both glucogenic and ketogenic. Leucine and lysine are the only totally ketogenic amino acids and upon catabolism generate acetyl-CoA.
The ketogenic amino acids are:
- Isoleucine
- Phenylalanine
- Threonine
- Tryptophan
- Tyrosine
- Leucine
- Lysine
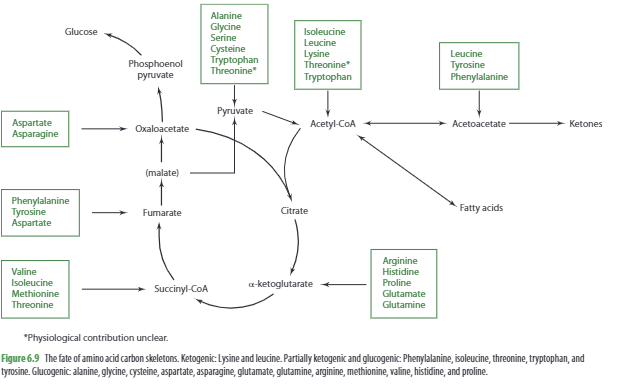
Lipid (Cholesterol & Fatty Acid) Production
The oxidation of several amino acids—including isoleucine, leucine, lysine, tryptophan, and threonine—yields acetyl-CoA, which can be metabolized to produce cholesterol. Leucine, however, is also the only amino acid whose catabolism directly generates b-hydroxy b-methylglutaryl-CoA, an intermediate in cholesterol synthesis. Moreover, leucine oxidation produces another metabolite, b-hydroxy b-methylbutyrate (HMB), which appears to promote de novo cholesterol synthesis in muscle, enabling cell growth and function.
In times of excess energy and protein intakes coupled with adequate carbohydrate intake, the carbon skeleton of amino acids may be used to synthesize fatty acids. Leucine’s carbon skeleton, for example, is used to synthesize fatty acids in adipose tissue.
Other Uses of Amino Acids
In addition to the above compounds that can be produced by the body by oxidizing amino acids, the compounds in the image below can also be produced.
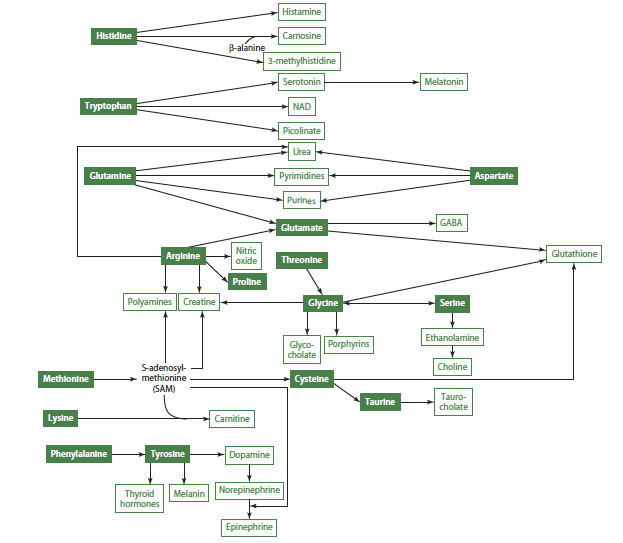
Protein Synthesis
After eating, anabolism increases in tissues, including protein synthesis, which requires both amino acids and energy. Fast proteins, such as whey protein, some soy proteins, amino acid mixtures, and protein hydrolysates, stimulate muscle protein and whole-body protein synthesis more effectively than slow proteins like casein. Slow proteins, however, are still important because they result in lower and more prolonged plasma amino acid concentrations, which reduces protein breakdown that occurs between meals and overnight.
Hormones play a significant role in amino acid utilization for protein synthesis. During prolonged fasting, protein synthesis still occurs but at a lower rate, while protein degradation predominates due to the release of epinephrine and cortisol, and a higher glucagon-to-insulin ratio in the blood. This ratio inhibits insulin’s ability to inhibit protein degradation and diminishes the overall rate of protein synthesis. However, glucagon promotes the hepatic synthesis of some proteins, such as enzymes for gluconeogenesis and ureagenesis, and cortisol promotes muscle protein catabolism and hepatic use of amino acids for gluconeogenesis and ureagenesis.
Insulin is anabolic, and it promotes protein synthesis and inhibits protein degradation. It is secreted in response to a rise in incretins, blood glucose, and some blood amino acid concentrations after food consumption. Insulin stimulates amino acid uptake into tissues by increasing the activity and transcellular movement of amino acid transporters. It also inhibits enzymes responsible for amino acid degradation. Insulin and leucine, which stimulates insulin secretion, also stimulate protein synthesis through different mechanisms.
Amino acids can regulate gene expression and promote changes in cell volume and protein synthesis through intracellular signaling pathways. Leucine and its metabolite HMB promote protein synthesis through increased phosphorylation of mTOR, which mediates the effects of insulin on protein synthesis but also exerts effects on protein synthesis that are independent of insulin.
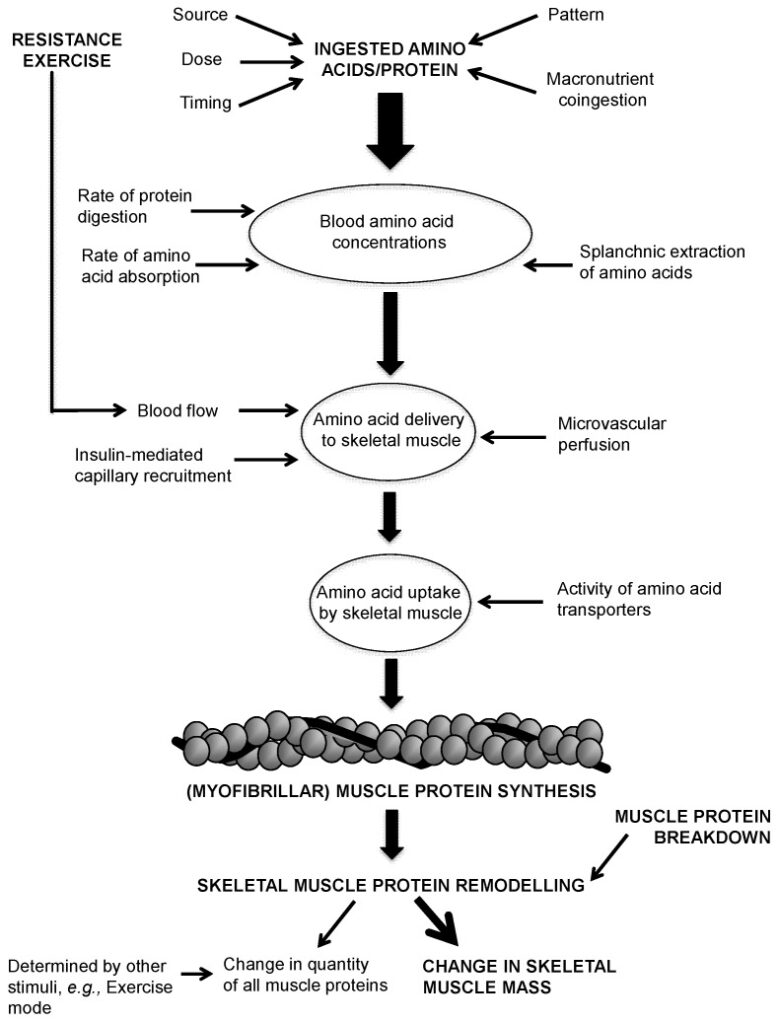
Tissue turnover is a constant process of breakdown and synthesis that maintains the amount of tissue in the body. For protein-based tissues like skeletal muscle and plasma proteins, this turnover involves continuous breakdown and resynthesis. If synthesis exceeds breakdown, there will be a net increase in the amount of that protein. Conversely, if breakdown exceeds synthesis, there will be an overall loss in the amount of that protein. If synthesis and breakdown are equal, there will be no long-term change in the amount of that protein.
For athletes seeking to build muscle, the goal is to ensure that skeletal muscle protein synthesis is either equal to or greater than protein breakdown. This can be achieved by increasing protein synthesis, decreasing protein breakdown, or both simultaneously.
Different tissues turn over at drastically varying rates. Plasma proteins made in the liver may turn over in a matter of hours while skeletal muscle protein may take days to turn over; tissues such as tendons and ligaments may take months or years to turn over completely.
The process of protein synthesis requires that amino acids are to be pulled out of the free pool for incorporation into the protein being synthesized. Protein breakdown releases amino acids back into the free pool. Protein turnover is energetically costly, and it’s been estimated that protein turnover may account for 15-25% of basal metabolic rate.
Protein turnover might seem as a wasteful process for the body to undergo, especially since the net result is more or less maintenance of bodily tissue, however, it appears to play a crucially important role in dealing with stressful situations by providing amino acids where they are needed.
Reduced protein turnover might compromise the body’s ability to rapidly deal with stressful stimuli (1, 2).
The amino acid content of a meal plays the major role in terms promoting protein synthesis, while insulin plays a secondary role. Assuming that sufficient amino acids are available, only very small amounts of maximal stimulation of protein synthesis via amino acids. Insulin also increases amino acid transport into skeletal muscle and some research suggests a direct role of insulin on protein synthesis.
Raising insulin levels without raising amino acid levels by consuming protein, has little to no effect on muscle protein synthesis. In fact, In fact, elevating insulin without simultaneously increasing amino acid availability tends to decrease protein synthesis, due to a decrease in circulating amino acid concentrations.
When it comes to protein breakdown, meal absorption appears to decrease protein breakdown via a combination of both increased amino acid availability (especially leucine), along with the increase in insulin.
Although insulin plays a minor role in promoting protein synthesis, insulin appears to play a primary role in decreasing protein breakdown.
Outside of the processes related to normal growth or aging, possibly the single greatest factor influencing skeletal muscle metabolism is training. Although the physiology of resistance and endurance training are significantly different, both impact profoundly on skeletal muscle metabolism. Resistance training affects both protein synthesis and breakdown with both being increased following training.
Resistance training has a net anabolic effect on the body, essentially “telling” it to maintain body protein stores at a higher level, eventually leading to increased levels of muscle mass.
Functional Roles of Protein
Proteins are an essential class of molecules found in living cells that perform a variety of functions. Proteins account for more than half of the solid content of cells, and their roles are quite diverse, so they are categorized according to their functions.
Some proteins have structural roles in the body. For example, actin and myosin are the two main contractile proteins found in cardiac, skeletal, and smooth muscles. Collagen, elastin, and keratin are fibrous proteins that are found in bone, teeth, skin, tendons, cartilage, blood vessels, hair, and nails.
Enzymes are protein molecules that act as catalysts, changing the rate of reactions occurring in the body. Enzymes are necessary for sustaining life and are found in the body both intracellularly and extracellularly. Enzymes combine selectively with other molecules (called substrates) in the cell, and the active site on the enzyme is where the enzyme and substrate bind to generate a product.
Some proteins require cofactors or coenzymes to carry out the reaction, and minerals such as zinc, iron, and copper function as cofactors for some enzymes. B vitamins serve as coenzymes for many enzymes.
Hormones are proteins that act as chemical messengers in the body, regulating metabolic processes, promoting enzyme synthesis, or affecting enzyme activity. Hormones are synthesized and secreted by endocrine tissue and transported in the blood to target tissues or organs, where they bind to protein receptors on membranes.
Proteins can serve as buffers in the body and help regulate acid-base balance. They can also influence fluid balance and attract and keep water inside a particular area, contributing to osmotic pressure.
Immunoprotection is provided to the body by a group of proteins called immunoproteins, also called immunoglobulins or antibodies. Immunoglobulins bind to antigens, typically foreign substances, such as bacteria or viruses that have entered the body, and create immunoprotein-antigen complexes that can be recognized and destroyed through reactions with complement proteins or cytokines.
Nitrogen-Containing-Non-Protein-Compounds
Amino acids, in addition to being used for protein synthesis, also play a crucial role in the synthesis of nitrogen-containing compounds in the body. These compounds include:
Glutathione: a tripeptide made from glycine, cysteine, and glutamate, and is a potent antioxidant that protects cells from damage caused by free radicals.
Carnitine: a nitrogen-containing compound made from lysine, primarily found in animal foods, and is essential for the transport of fatty acids into the mitochondria for energy production. A deficiency in carnitine can lead to impaired energy metabolism.
Creatine: a key component of creatine phosphate, which is used as a storehouse for high-energy phosphate in muscles, and can be obtained from food or synthesized from arginine, glycine, and methionine. Creatine is essential for muscle contraction and energy metabolism.
Carnosine: synthesized from histidine and beta-alanine, primarily found in skeletal and cardiac muscles, and may act as a buffer to help prevent muscle fatigue.
Choline: synthesized in the liver from phosphatidylethanolamine and used to make phosphatidylcholine and sphingomyelin, which are important components of cell membranes. Choline is also used to make the neurotransmitter acetylcholine and is found in foods like eggs, liver, and legumes.
Purine and pyrimidine bases: nitrogenous bases that provide the source of nitrogen for the synthesis of DNA and RNA, and are derived from amino acids in the body. These bases can be divided into two categories: pyrimidines and purines.
Glutamine Metabolism
Glutamine is a crucial amino acid with several important functions in the human body. In the intestines, it serves as a source of energy and stimulates the growth of gastrointestinal mucosa cells, preventing atrophy and bacterial translocation. Glutamine also enhances the synthesis of heat shock proteins and is needed for the production of mucins in mucus secretions within the GI tract. Due to these roles, many nutrition products are enriched with glutamine.
The human GI tract utilizes up to 10 g of glutamine per day, while immune cells require over 10 g per day. Glutamine produced by skeletal muscles and other tissues is also taken up by intestinal cells. Additionally, glutamine is involved in ammonia transport, with glutamine synthetase catalyzing the conversion of ammonia or ammonium ions into glutamine in extrahepatic tissues. The body produces an estimated 40-80 g of glutamine per day.
During hypercatabolic conditions such as infection and trauma, the demand for glutamine increases significantly, potentially depleting stores and impairing cellular functions. Therefore, adequate glutamine intake is especially critical during illness and injury.
The Alanine-Glucose Cycle
In addition to glutamine, the amino acid alanine is also important in the intertissue (between tissues) transfer of amino groups generated from amino acid catabolism. These reactions are known as the alanine-glucose cycle detailed in the diagram below. This cycle allows the body to produce significant amounts of glucose without requiring dietary carbohydrate (gluconeogenesis).
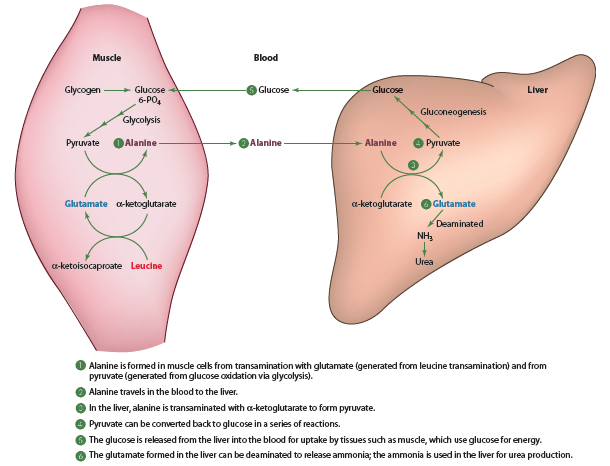
Skeletal Muscle
Skeletal muscle makes up a significant portion of the body’s mass, accounting for up to 43%. After consuming a meal, amino acid uptake by skeletal muscles increases, resulting in net protein synthesis. However, in a postabsorptive state, such as between meals or during fasting, muscle protein degradation exceeds synthesis, leading to the release of amino acids into the bloodstream. Exercise-induced stress triggers cortisol secretion, which promotes muscle and amino acid catabolism.
Certain amino acids, such as aspartate, asparagine, glutamate, leucine, isoleucine, and valine, are preferentially catabolized by skeletal muscles compared to other tissues. Leucine’s ability to stimulate protein synthesis has led to its use in branched-chain amino acid supplements by some athletes.
Plasma amino acid concentrations do not reflect changes in muscle mass. Instead, creatinine and 3-methylhistidine are used as indicators of muscle mass and degradation, respectively. Strength-trained individuals may have elevated creatinine levels due to their increased muscle mass. Unfortunately, doctors sometimes misinterpret this as a sign of inflammation, kidney malfunction, or muscle trauma.
How Much Protein Do You Need?
You may have heard the common belief among bodybuilders that consuming 1g of protein per pound of bodyweight (or 2.2g per kilogram) is essential for building muscle and losing fat. However, despite decades of research, there is still no definitive consensus on the optimal protein intake for athletes, as well as for the average non-training individual. The ongoing debate among researchers and the existence of conflicting studies only add to the confusion and lack of agreement on the matter.
It’s important to note that when discussing protein requirements, we are specifically referring to athletes and not the elderly or non-athletic individuals.
For older people there is an anabolic resistance that occurs with aging, making their protein requirements systematically higher than younger individuals.
When the IIFYM craze hit the fitness industry back in the early 2010s, that magical 1g/lb number decreased to 0.82g/lb, and depending on the study that was cited, went down as low as 0.67g/lb. But what is the truth? Should you gulp down scoop after scoop of whey protein powders or eat chicken breast after chicken breast or should you not care at all since protein needs are over-exaggerated anyways?
Studies show that there are no additional benefits for muscle gain when going higher than 1g/lb in non dieting conditions, assuming adequate calorie intake. When dieting however, protein requirements go up and can be especially high for lean athletes.
Looking at weight and fat loss in general, eating insufficient dietary protein may cause people to over eat in general (presumably the body increases hunger in an attempt to get sufficient protein), contributing to obesity in the modern world.
After a fat loss diet has ended, higher protein intakes have been shown to not only help prevent weight regain but, especially when resistance training is being done, to cause more of any weight that is regained to be from LBM. Similarly, a high protein intake is absolutely crucial for the optimization of muscle mass retention during weight loss diets.
The combination of sufficient protein intake with proper resistance training also has the additive effect of improving body composition[1].
The problem with most studies is that generally they have not examined performance as an endpoint. They only address issues of protein requirements. Coaches and athletes are ultimately less interested in scientific arguments and more interested in what will optimize athletic performance.
Also, the definition “requirement” is context specific. The requirements of a strength athlete trying to make weight versus a strength/power athlete wanting to gain strength and mass or an endurance athlete that wants to avoid muscle mass gains while supporting performance adaptations will all be completely different. Answering the question of “How much protein is required?” depends entirely on the context.
Additionally, while small changes in either muscle mass or performance may not be statistically significant in research terms, those same small changes may be crucially important in high level sports. While a 1% difference in gains means nothing in a statistical or scientific sense, it can make the difference between first and last place in the real world of high-level sports.
This study, took all the points I mentioned above, examined both sides of the low protein and high protein arguments in some
detail and concluded that a daily protein intake of 2.5-3.0 g/kg (1.1-1.4 g/lb) for strength/power athletes is not harmful, may give small but important performance improvements over the long-term, more than covering any needs for protein synthesis, and any excess will simply be oxidized off in the first place.
Protein has other additional and important qualities like its superior effect on satiety over carbs and fat, ensuring good diet compliance, since you won’t get hungry and risk overeating. Secondly, TEF (Thermic Effect of Food) is much greater for protein than for both carbs and fat. Due to this, Livesey proposed that protein should be counted as 3.2 kcal and not 4 kcal as the current guidelines state.
This review concluded that when comparing two hypo-energetic diets with the same caloric intake, the diet with the highest protein percentage of total calorie intake will show superior results due to protein’s effects on thermogenesis and satiety. It is precisely this protein induced thermogenesis that helps you lose body weight faster when dieting, while keep you leaner during mass gaining phases. Another added benefit of higher protein intakes is that they make you think about your food choices more, therefore making you cheat less.
As an athlete you have to make sure that you provide your body with a sufficient protein intake. Eating 1-1.4 g/lb (2.2-3.0 g/kg) of body weight will help you reap all the benefits of higher protein intakes that were discussed previously, while optimizing your performance to supreme levels.
Protein Myths
While individuals with compromised kidney function may have to reduce their protein intake and there’s no evidence suggesting that protein harms the kidneys.
In fact, increased dietary protein intake may actually improve kidney function and there is no evidence that a high-protein intake has any effect unless there is a pre-existing condition. There has never been a case of kidney damage linked to protein intake and studies using high protein intakes to athletes show no negative effects.
It’s also often stated that a high protein intake is harmful to bone health but this is only true if insufficient calcium/Vitamin D along with fruits and vegetables are being consumed. When those nutrients are consumed in adequate amounts, a higher protein intake actually improves bone density.
In the modern Western diet, high meat intakes are typically associated with a low fruit and vegetable intake and it is the lack of fruits and vegetables that are more to blame than the presence of meat itself.
Lean red meat has been shown to improve health, is inversely associated with mortality, contains many cancer preventing nutrients, and does NOT influence cardiovascular disease risk factors.
Meal Frequency
Regardless of potential health benefits related to meal frequency, there are practical reasons to eat more or less frequently and ultimately it will depend on individual preferences, tolerance, lifestyle and total caloric intake. For athletes with high caloric requirements, eating multiple smaller meals may make consuming sufficient calories (and especially carbohydrates) easier to accomplish compared to eating a few larger meals.
Taking a caloric intake of 4000 kcals for example it may be easier to divide into 4 meals of 1000 kcals each instead of 2 large 2000 kcal meals. Similarly a demale that has to eat 1200-1500 kcals will most likely find it easier to adhere to fewer larger meals over 200 kcal “meals”. Another issue has to do with the amount of training being done. An athlete that trains for 3-4 hours per day, for example a cyclist, or an athlete that trains twice per day, have a limited amount of time to consume a large number of calories, thus eating fewer larger meals may be the only realistic way to consume sufficient calories to support a heavy training schedule.
Work schedules may or may not allow athletes to fit in multiple small meals per day. So even if such an eating schedule is ideal, it may not be practically possible. From an evolutionary stand point, “Our ancestors consumed food much less frequently and often had to subsist on one large meal per day, and thus from an evolutionary perspective, human beings were adapted to intermittent feeding rather than to grazing”, so that should also be kept into account.
Summary
Tldr; Proteins are formed by amino acids, which vary in their chemical structure. The chemical structure of an amino acid determines its function in the body. Dietary protein is your body’s principle source of amino acids. Protein is generally digested in the stomach, subsequently absorbed in the intestine and then transported in the blood to the liver.
Protein synthesis as well as catabolism occur in the body at various locations across the entire day. Net protein balance determines whether there is net tissue build-up or breakdown. Which tissues are built on and which are broken down is determined by many factors, notably hormone levels.
Contractile proteins allow you to flex your muscles. Fibrous proteins give strength to your connective tissue.
Enzymes are proteins that speed up or slow down the chemical reactions and processes in the body.
Certain proteins are hormones that act as messengers in the body activating or deactivating other processes. Some proteins act as buffers that regulate acidity levels, while other proteins act as fluid balancers regulating water levels, or as transporters, sending nutrients, hormones or drugs to and within the body. There are also some proteins that are anti-bodies in your immune system preventing you from getting sick, while other proteins have similar functions to keep you healthy when you do get unwell.
Glutamine is particularly important for intestinal health and your immune system.
Creatinine levels correlate roughly with muscle mass whereas 3-methylhistidine levels in your urine are a rough marker of protein catabolism in your body. Strength trained individuals often have elevated creatinine levels. Since creatinine levels correlate roughly with muscle mass, many doctors mistakenly interpret this as a sign of inflammation, kidney malfunction or muscle trauma.
Protein requirements vary. During weight loss diets, higher protein intakes are required in order to retain Lean Body Mass (LBM). Protein has additional benefits including improved body composition and satiety, higher Thermic Effect of Food (TEF), as well as help prevent weight regain. A higher protein intake will also make you think about your food choices more, making you cheat less. As an athlete you have to make sure that you provide your body with a sufficient protein intake. Eating 1-1.4 g/lb (2.2-3.0 g/kg) of body weight will help you reap all the benefits of higher protein intakes that were discussed previously, while optimizing your performance to supreme levels.
Protein is NOT harmful to your kidneys, bone health and density. In fact, it’s quite the opposite. You need protein in order to be healthy.
Meal frequency is completely individualistic, based on the athlete’s work schedule, training duration and frequency, as well as preferences and ability to tolerate and digest larger meals during higher caloric intakes. Fewer larger meals may be more beneficial for lower caloric intakes.